Chapter 12: Respiratory care, CPR and blood transfusion
Skip chapter table of contents and go to main content

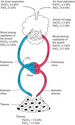
Anatomy and physiology
The respiratory system is a complex system that is responsible for the efficient delivery and exchange of respiratory gases, primarily oxygen (O2) and carbon dioxide (CO2). The respiratory system is composed of the following structures, some of which are illustrated in Figure 12.1:
- the respiratory centres in the medulla oblongata and pons of the brain
- the nose, mouth and connecting airways
- the trachea, main bronchus, bronchioles and alveoli
- the respiratory muscles (diaphragm and intercostal muscles)
- the respiratory nerves (subphrenic and intercostal nerves)
- the bone structures of the thorax (ribs, vertebrae and sternum)
- the lung parenchyma
- the pleura and pleural fluid (Tortora and Derrickson [272]).

Figure 12.1 The major respiratory organs in relation to surrounding structures.
Source: Marieb and Hoehn ([146]).
Alteration, damage or blockage to any of the structures listed above may result in respiratory impairment or failure. It is essential when considering respiratory function to remember the close association between the cardiovascular, neurological, musculoskeletal and respiratory systems (Marieb and Hoehn [147]).
Tissue oxygenation
Every cell of the body requires a continuous supply of oxygen for the growth and repair of tissues, and to ensure optimum metabolism. Oxygen is drawn into the body through the nose and mouth; it then travels down the trachea and into the smaller airways and alveoli of the lungs. Once it has reached the alveoli, oxygen is able to diffuse into the network of capillaries that surround the lung. The oxygenated blood travels via the pulmonary vein from the lungs to the heart, where it is then pumped to all cells of the body via the arterial system (Tortora and Derrickson [272]). ‘Hypoxia’ is the term used to describe a lack of tissue oxygenation.
Within the cell mitochondria, oxygen and glucose are used to produce energy in the form of adenosine triphosphate (ATP), and carbon dioxide is produced as a waste product. This is known as aerobic ‘cellular respiration’. In low‐oxygen conditions, cellular respiration becomes anaerobic, generating less ATP and producing the by‐product lactic acid. If the low‐oxygen state is allowed to continue, lactic acid will accumulate, leading to metabolic acidosis and cell death (Lenihan and Cormac [132]).
There are three components of cellular oxygenation: oxygen uptake, oxygen transportation and oxygen utilization. Oxygen uptake is the process of extracting oxygen from the environment. Oxygen transportation is the mechanism by which the uptake of oxygen results in the delivery of oxygen to the cells. Oxygen utilization is the metabolic need for molecular oxygen by the cells of the body (Tortora and Derrickson [272]).
Oxygen uptake
The air that we breathe in from the atmosphere is composed of the following gases:
- oxygen: 21%
- carbon dioxide: 0.03%
- nitrogen: 78%
- argon: >0.9%
- other gases: 0.07%.
Inspired air at sea level has a total atmospheric pressure of 101.3 kPa (760 mmHg). According to Dalton's law, where there is a mixture of gases, each gas exerts its own pressure as if there were no other gases present (Jones and Berry [120]). The pressure of an individual gas in a mixture is called the ‘partial pressure’ and is denoted as Pa. This is followed by the symbol of the gas. For example, the partial pressure of oxygen is written as PaO2 (Tortora and Derrickson [272]). The partial pressures of the three main gases in the air are as follows:
- oxygen: 0.21 × 101.3 = 21.3 kPa (159.8 mmHg)
- carbon dioxide: 0.03 × 101.3 = 3.0 kPa (22.8 mmHg)
- nitrogen: 0.78 × 101.3 = 79.0 kPa (592.6 mmHg)
The partial pressure controls the movement of oxygen and carbon dioxide between the atmosphere and the lungs, the lungs and the blood, and finally the blood and the cells.
Gaseous exchange
Gases move by diffusion. Diffusion is the movement of gas molecules from an area of relatively high partial pressure to an area of low partial pressure (Tortora and Derrickson [272]). Oxygen diffuses from the alveoli, where the partial pressure is highest, into the pulmonary capillaries, where it is lower. From here it continues to travel, and as the partial pressure changes it diffuses into the tissues and finally the mitochondria of the cells. The waste product carbon dioxide diffuses in the same way back from the cells to the alveoli, where the partial pressure of carbon dioxide is lowest (Figure 12.2).
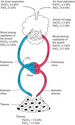
Figure 12.2 Gas movement in the body is facilitated by partial pressure differences. Top of figure illustrates pressure gradients that facilitate oxygen (O2) and carbon dioxide (CO2) exchange in the lungs. Bottom of figure shows pressure gradients that facilitate gas movements from systemic capillaries to tissues. LA, left atrium; LV, left atrium; RA, right atrium; RV, right ventricle.
Inspired air encounters water vapour as it is warmed and humidified in the upper airways of the respiratory tract. Water vapour exerts its own partial pressure of 6.3 kPa (47 mmHg), which must be subtracted from the total atmospheric pressure to give the corrected total atmospheric pressure (Brady [29]):
- corrected total atmospheric pressure: 101.3 – 6.3 = 95 kPa (713 mmHg)
This figure of 95 kPa is then used to find the partial pressures of each gas:
- oxygen: 0.21 × 95 = 20.0 kPa (150.0 mmHg)
- carbon dioxide: 0.03 × 95 = 2.9 kPa (21.8 mmHg)
As oxygen continues to pass down the respiratory tract to the alveoli, it encounters carbon dioxide leaving the respiratory tract. This exerts a partial pressure of approximately 5.3 kPa (40.0 mmHg). This in turn must be subtracted to determine the correct value for oxygen (Jones and Berry [120], O'Driscoll et al. [209]). For example:
- corrected partial pressure of oxygen: 20.0 kPa (150.0 mmHg) – 5.3 kPa (40.0 mmHg) = 14.7 kPa (110 mmHg)
Oxygen transportation
Oxygen is carried in the blood in two ways:
- Dissolved in the plasma (serum): only 2–3% (0.03 mL O2 per 100 mL of plasma) is carried in this way as oxygen is not very soluble (Marieb and Hoehn [147]). It is expressed as the PaO2.
- Bound to the haemoglobin of the red blood cells: 95–98% of oxygen is carried in this way; it is measured as the percentage of haemoglobin saturated with oxygen. Each gram of haemoglobin can carry 1.34 mL of oxygen per 100 mL blood (Thomas and Lumb [269]). It is expressed as the SaO2 when measured from arterial blood gas. When pulse oximetry is used, it is expressed as SpO2.
Haemoglobin is composed of haem (iron) and globulin (protein). Each haemoglobin molecule has four binding sites, each able to carry one molecule of oxygen. A haemoglobin molecule is said to be fully saturated when oxygen is bound to all four molecules. When fewer than four are attached, the haemoglobin is said to be partially saturated (Jones and Berry [120]). The bond between haemoglobin and oxygen is represented by the oxygen disassociation curve and is affected by various physiological factors that shift the curve to the right or left (Figure 12.3).
Oxyhaemoglobin curve shift to the right
When a shift occurs to the right, there is reduced binding of oxygen to haemoglobin and oxygen is given up more easily to the tissues. Oxygen saturation of the haemoglobin molecule will be lower (Collins et al. [49], Kaufman and Dhamoon [124]). Increases in the following cause the curve to shift to the right:
- body temperature (due to infection or sepsis)
- hydrogen ion content (acidaemia), known as the Bohr effect (due to sepsis or other shock conditions)
- carbon dioxide (due to sepsis or pulmonary disease)
- 2,3‐diphosphoglycerate (2,3‐DPG) (an enzyme found in the red bloods cells that affects haemoglobin and oxygen binding).
Oxyhaemoglobin curve shift to the left
When a shift occurs to the left, there is an increase in the binding of oxygen to the haemoglobin, oxygen is given up less easily to the tissues and cellular hypoxia can occur (Jones and Berry [120], Kaufman and Dhamoon [124]). Decreases in the following cause the curve to shift to the left:
- body temperature (due to exposure to cold, near drowning or trauma)
- hydrogen ion content (due to alkalaemia)
- carbon dioxide (due to hyperventilation)
- 2,3‐DPG.
Oxygen utilization
Oxygen uptake in the lungs is shown by the upper flat part of the curve. When the PaO2 is between 8.0 and 13.3 kPa (60–100 mmHg), the haemoglobin molecule is saturated with more than 90% oxygen. At this point of the curve, large changes in the PaO2 lead to small changes in the SaO2 because the haemoglobin is almost completely saturated. The lower part of the curve shows the release of oxygen from the haemoglobin molecule to the tissues. Oxygen is given up very easily to the tissues and small changes in the PaO2 cause major changes to the SaO2 (Marieb and Hoehn [147]). A patient's PaO2 must therefore be kept above 8 kPa (60 mmHg) to prevent hypoxia and cell death (Jones and Berry [120]). ‘Hypoxaemia’ is the term used to describe a low partial pressure of oxygen within the blood.
In addition to measuring the partial pressure of oxygen within the arterial blood, the fraction of inspired oxygen is also considered (FiO2). This represents the percentage of oxygen participating in gas exchange. Room air has a FiO2 of 0.21 (21%); therefore, any additional oxygen inhaled will increase the FiO2. If a patient is receiving large concentrations of oxygen therapy and is hypoxaemic, this may indicate a problem within the lungs caused by a ventilation/perfusion mismatch, a shunt, alveolar hypoventilation, decreased partial pressure of oxygen or decreased diffusion (Sarkar et al. [242]).
Oxygen consumption
At rest, the normal oxygen consumption rate is approximately 200–250 mL per minute. As the available oxygen per minute in an average‐sized man is about 700 mL, this means there is an oxygen reserve of 450–500 mL per minute (MacIntyre [140]). Factors that increase the consumption of oxygen include fever, sepsis, shivering, restlessness and increased metabolism (Semenza [249]). It is difficult to say at which absolute level oxygen therapy is necessary, as each situation should be judged by the clinical condition of the patient and their oxygen requirements. Generally speaking, additional oxygen will be required when the PaO2 has fallen to 8 kPa (60 mmHg) or less (O'Driscoll et al. [209]), as measured on an arterial blood gas sample.
Carbon dioxide excretion
The second function of the respiratory system is to excrete carbon dioxide from the lungs during expiration in order to maintain a normal level of carbon dioxide in the blood (4.5–6.0 kPa or 34–45 mmHg). Carbon dioxide has a direct effect on the respiratory centre in the brain (Marhong and Fan [145]). As the carbon dioxide level rises and diffuses from the blood into the cerebrospinal fluid (CSF), it is hydrated and carbonic acid is formed. The acid then dissociates and hydrogen ions are liberated. Because there is no bicarbonate in the CSF to buffer the hydrogen ions, the pH of the CSF falls, which excites the central chemoreceptors, resulting in an increased respiratory rate (Cheng and Jusof [43], Marieb and Hoehn [147]).